Bioelectrochemical systems: Difference between revisions
Created page with "Category:Technologies & Solutions Bioelectrochemical systems (BESs) are systems integrated systems combining wastewater treatment with energy production and resource r..." |
mNo edit summary |
||
(3 intermediate revisions by 3 users not shown) | |||
Line 1: | Line 1: | ||
[[Category:Technologies & Solutions]] | [[Category:Technologies & Solutions]] | ||
[[Bioelectrochemical systems]] (BESs) are | [[Bioelectrochemical systems]] (BESs) are integrated systems combining wastewater [[treatment]] with energy production and resource [[recovery]]. They utilize microorganisms to biochemically catalyse complex substrates into useful energy products, in which the catalytic reactions take place on electrodes<ref>[https://www.sciencedirect.com/science/article/pii/B9780128111574000073 Sustainable Waste-to-Energy Technologies: Bioelectrochemical Systems]</ref>. | ||
BESs have boomed over the past decade for their contribution as an emerging sustainable technology for concurrent electricity production and wastewater treatment. In addition, BESs also offer unique possibilities for clean and efficient production of fuels and high-value chemicals using microorganisms. Applications of BESs include: microbial desalination cells (MDCs), sediment/plant microbial fuel cells (MFCs), microbial electrosynthesis (MES) converts | BESs have boomed over the past decade for their contribution as an emerging sustainable technology for concurrent electricity production and wastewater [[treatment]]. In addition, BESs also offer unique possibilities for clean and efficient production of fuels and high-value chemicals using microorganisms. Applications of BESs include: microbial desalination cells (MDCs), sediment/plant microbial fuel cells (MFCs), microbial electrosynthesis (MES) converts CO<sub>2</sub> or organic molecules to higher value organic molecules, they are efficient bioreactors for the treatment of pollutants and toxic wastewater (process known as bioelectrochemical treatment (BET)/microbial electroremediation (MER)). | ||
In these electrochemical systems, the redox potentials of an oxidation reaction at the anode and a reduction reaction at the cathode create a potential difference which is the driving force for electrons to flow from a low potential to high potential. This flow of electrons through an external circuit is measured as electric current. Whenever, microbes or enzymes are involved in the oxidation or reduction or both reactions, the system is termed as a bioeclectrocehmical system (BES) or microbial electrochemical system (MXC)<ref name="ref1">[https://www.sciencedirect.com/science/article/pii/S0960148116301860 Bioelectrochemical Systems (BESs)]</ref>. | In these electrochemical systems, the redox potentials of an oxidation reaction at the anode and a reduction reaction at the cathode create a potential difference which is the driving force for electrons to flow from a low potential to high potential. This flow of electrons through an external circuit is measured as electric current. Whenever, microbes or enzymes are involved in the oxidation or reduction or both reactions, the system is termed as a bioeclectrocehmical system (BES) or microbial electrochemical system (MXC)<ref name="ref1">[https://www.sciencedirect.com/science/article/pii/S0960148116301860 Bioelectrochemical Systems (BESs)]</ref>. | ||
=== Types of Bioelectrochemical Systems === | === Types of Bioelectrochemical Systems === | ||
[[File:Bioelectrochemical Systems Graphic.png|right|Schematic overview of various types of bioelectrochemical systems (BESs). All Rights Reserved.]]<ref name="ref1" /> | |||
# Microbial fuel cells (MCFs) | # Microbial fuel cells (MCFs) | ||
# Microbial electrolysis cells (MECs) | # Microbial electrolysis cells (MECs) | ||
Line 15: | Line 16: | ||
# Microbial desalination cells (MDCs) | # Microbial desalination cells (MDCs) | ||
<br clear=all /> | <br clear=all /> | ||
==== 1. Microbial fuel cells (MCFs) ==== | |||
[[File:MFC Diagram.png|right|MFC Diagram. All Rights Reserved.]] | |||
MFCs harness electrical current from the microbial oxidation of [[Organic Waste|organic matter]] using a solid electrode as an electron acceptor. The anode surface facilitates microbial attachment and oxidation of organics, therefore generating electrons which are then simultaneously transferred to the cathode compartment through an external circuit containing an external load. Electroneutrality is warranted by ions transport through an ion-permeable medium or a membrane while electricity is produced in the process. | |||
1. Microbial fuel cells (MCFs) | |||
MFCs harness electrical current from the microbial oxidation of organic matter using a solid electrode as an electron acceptor. The anode surface facilitates microbial attachment and oxidation of organics, therefore generating electrons which are then simultaneously transferred to the cathode compartment through an external circuit containing an external load. Electroneutrality is warranted by ions transport through an ion-permeable medium or a membrane while electricity is produced in the process. | |||
A number of bacterial species have been identified with the ability to produce electric current. The Shewanella and Geobacter species were earlier found as the electron-transferring microbes and so are well-studied. The presence of Geobacteraceae in the bioanode community often showed high power densities registered in MFCs. | A number of bacterial species have been identified with the ability to produce electric current. The Shewanella and Geobacter species were earlier found as the electron-transferring microbes and so are well-studied. The presence of Geobacteraceae in the bioanode community often showed high power densities registered in MFCs. | ||
The performance of MCFs relies on electrode material and its structure. Graphite electrodes with a roughened surface have shown to produce higher power densities than flat graphite electrodes. Increasing the surface area of MFC anodes using porous materials such as graphite brush, carbon felt and carbon nanotubes, have shown considerable increase in current density. Higher surface area of electrodes provides a larger surface for bacterial adhesion and electron transfer between the bacteria and electrode. Direct electron transfer phenomenon of microbes with metal electrodes has also been demonstrated including dimensionally stable anodes (DSA, titanium over iridium and tantalum oxide), stainless steel and platinum electrodes. However, current densities obtained with porous carbon anodes are typically higher than those reported with metals except for platinum<ref name="ref1" />. | The performance of MCFs relies on electrode material and its structure. Graphite electrodes with a roughened surface have shown to produce higher power densities than flat graphite electrodes. Increasing the surface area of MFC anodes using porous materials such as graphite brush, carbon felt and carbon nanotubes, have shown considerable increase in current density. Higher surface area of electrodes provides a larger surface for bacterial adhesion and electron transfer between the bacteria and electrode. Direct electron transfer phenomenon of microbes with metal electrodes has also been demonstrated including dimensionally stable anodes (DSA, titanium over iridium and tantalum oxide), stainless steel and platinum electrodes. However, current densities obtained with porous carbon anodes are typically higher than those reported with metals except for platinum<ref name="ref1" />. | ||
<br clear=all /> | <br clear=all /> | ||
<ref>[https://letstalkscience.ca/educational-resources/stem-in-context/microbial-fuel-cells MFC Diagram]</ref> | <ref>[https://letstalkscience.ca/educational-resources/stem-in-context/microbial-fuel-cells MFC Diagram]</ref> | ||
==== 2. Microbial electrolysis cells (MECs) ==== | |||
[[File:MEC Diagram.png|right|MEC Diagram. All Rights Reserved.]] | |||
MECs utilize the property of bacteria to convert chemical energy to electrical energy and allow electrolysis of water. External power applied onto the electrical circuit of BES drives electrons from anode to the cathode. This also supports the hydrogen production at the cathode which operates under [[Anaerobic Digestion|anaerobic]] conditions. However the anoxic environment in MECs, along with high concentrations of hydrogen production, can also promote methane production once CO<sub>2</sub> and methanogens are available. Methods to mitigate toxic build up includes the aeration of the cathode chamber between batches, lowering of the pH, operation at short retention times, giving a heat shock to the inoculum and adding chemicals that inhibit the growth of methanogens. | |||
MECs utilize the property of bacteria to convert chemical energy to electrical energy and allow electrolysis of water. External power applied onto the electrical circuit of BES drives electrons from anode to the cathode. This also supports the hydrogen production at the cathode which operates under anaerobic conditions. However the anoxic environment in MECs, along with high concentrations of hydrogen production, can also promote methane production once | |||
In an MEC with bioanode and biocathode, expensive metals such as platinum are not required as catalyst and the enrichment of microbes on the carbon cathode decreases the start-up time and produces comparable current densities to those of bioanode. Also the hydrogen synthesized in MECs can also drive the biochemical production of other chemicals<ref name="ref1" />. | In an MEC with bioanode and biocathode, expensive metals such as platinum are not required as catalyst and the enrichment of microbes on the carbon cathode decreases the start-up time and produces comparable current densities to those of bioanode. Also the hydrogen synthesized in MECs can also drive the biochemical production of other chemicals<ref name="ref1" />. | ||
<br clear=all /> | <br clear=all /> | ||
<ref>[https://www.sciencedirect.com/science/article/pii/S1110016815001635 MEC Diagram]</ref> | <ref>[https://www.sciencedirect.com/science/article/pii/S1110016815001635 MEC Diagram]</ref> | ||
==== 3. Microbial electrosynthesis (MES) ==== | |||
[[File:MES Diagram.png|right|MES Diagram. All Rights Reserved.]] | |||
MES (also known as bioelectrosynthesis) uses the reducing power generated from the anodic oxidation to produce value added products at the cathode. Cathodic biocatalysts (with attached cathodic biofilms) reduce the available terminal electron acceptor to produce value added products. Biocathodes are the key components of microbial electrosynthesis, where the electrode oxidizing microorganisms are involved in the formation of reduced value-added product such as acetate, ethanol, butyrate. MES includes the production of chemical compounds in an electrochemical cell by electricity-driven CO<sub>2</sub> reduction as well as reduction/oxidation of other [[Organic Waste|organic feedstocks]] using microbes as biocatalyst<ref name="ref1" />. | |||
<br clear=all /> | <br clear=all /> | ||
<ref>[https://www.researchgate.net/figure/Principle-of-microbial-electrosynthesis-at-the-cathode_fig2_308527483 MES Diagram]</ref> | <ref>[https://www.researchgate.net/figure/Principle-of-microbial-electrosynthesis-at-the-cathode_fig2_308527483 MES Diagram]</ref> | ||
==== 4. Enzymatic fuel cells (EFCs) ==== | |||
4. Enzymatic fuel cells (EFCs) | [[File:EFC Diagram.png|right|Schematic of an EFC-based Biosensor and its Detection Mechanism. All Rights Reserved.]] | ||
EFCs utilize specific enzymes on the electrode surface (or in electrolyte suspension) that facilitate the catalytic oxidation of fuel and drive specific reactions desired for various applications. Different enzymes are used on anode and cathodes, based on their specific redox function. As highly selective enzymes are used at each node, then this eliminates the need of any membrane between the anode and cathode compartments. Enzymatic catalysts usually oxidize the fuel partially and heat generation occurs as a result of side reactions which can impact enzymatic activity. The shelf life of these enzyme-based systems can be extended through encapsulation in micelle polymers that provide a buffering capacity for pH control and a hydrophobic niche to prevent enzymatic degradation<ref name="ref1" />. | EFCs utilize specific enzymes on the electrode surface (or in electrolyte suspension) that facilitate the catalytic oxidation of fuel and drive specific reactions desired for various applications. Different enzymes are used on anode and cathodes, based on their specific redox function. As highly selective enzymes are used at each node, then this eliminates the need of any membrane between the anode and cathode compartments. Enzymatic catalysts usually oxidize the fuel partially and heat generation occurs as a result of side reactions which can impact enzymatic activity. The shelf life of these enzyme-based systems can be extended through encapsulation in micelle polymers that provide a buffering capacity for pH control and a hydrophobic niche to prevent enzymatic degradation<ref name="ref1" />. | ||
<br clear=all /> | <br clear=all /> | ||
<ref>[https://www.mdpi.com/2079-6374/8/1/11/htm Schematic of an EFC-based Biosensor and its Detection Mechanism]</ref> | <ref>[https://www.mdpi.com/2079-6374/8/1/11/htm Schematic of an EFC-based Biosensor and its Detection Mechanism]</ref> | ||
5. Microbial solar cells (MSCs) | ==== 5. Microbial solar cells (MSCs) ==== | ||
[[File:MSC Diagram.png|right|MSC Diagram. All Rights Reserved.]] | |||
MSCs make use of photoautotrophic microbes or higher plants to entrap solar energy which is further utilized by electroactive bacteria to perform electrode-driven reactions. These reactions include generation of electric current or fuels such as hydrogen, methane and ethanol. Primarily photosynthesis leads to the generation of organic compounds, which are subsequently fed into the anode compartment where they are oxidized by electroactive microbes to produce electrons. These electrons are then transferred to the cathodic side where reduction of oxygen leads to the formation of water<ref name="ref1" />. | MSCs make use of photoautotrophic microbes or higher plants to entrap solar energy which is further utilized by electroactive bacteria to perform electrode-driven reactions. These reactions include generation of electric current or fuels such as hydrogen, methane and ethanol. Primarily photosynthesis leads to the generation of organic compounds, which are subsequently fed into the anode compartment where they are oxidized by electroactive microbes to produce electrons. These electrons are then transferred to the cathodic side where reduction of oxygen leads to the formation of water<ref name="ref1" />. | ||
<br clear=all /> | <br clear=all /> | ||
<ref>[https://www.sciencedirect.com/science/article/pii/S0167779910001757 MSC Diagram]</ref> | <ref>[https://www.sciencedirect.com/science/article/pii/S0167779910001757 MSC Diagram]</ref> | ||
==== 6. Plant microbial fuel cells (PMFCs) ==== | |||
6. Plant microbial fuel cells (PMFCs) | [[File:PMFC Diagram.png|right|Model of a PMFC Producing Electricity and Driving a Light Source. All Rights Reserved.]] | ||
PMFCs incorporate the living plant's root system into the MFC anode. The organic products (rhizodeposits) released to the soil by the roots are used as substrate for electricity production by electroactive microbes. So overall PMFCs harness solar radiation by transforming it into green electricity in a clean and efficient manner. The choice of a plant is important as it directly controls the amount of rhizodeposits for bioelectricity generation. PMFCs from Pennisetum setaceum are the most sustainable in terms of power production and S. anglica PMFCs with integrated oxygen reducing biocathodes reached the highest long-term power output. Reed manna grass, rice plants and Spartina anglica have also been used in separate studies<ref name="ref1" />. | PMFCs incorporate the living plant's root system into the MFC anode. The organic products (rhizodeposits) released to the soil by the roots are used as substrate for electricity production by electroactive microbes. So overall PMFCs harness solar radiation by transforming it into green electricity in a clean and efficient manner. The choice of a plant is important as it directly controls the amount of rhizodeposits for bioelectricity generation. PMFCs from Pennisetum setaceum are the most sustainable in terms of power production and S. anglica PMFCs with integrated oxygen reducing biocathodes reached the highest long-term power output. Reed manna grass, rice plants and Spartina anglica have also been used in separate studies<ref name="ref1" />. | ||
<br clear=all /> | <br clear=all /> | ||
<ref>[https://onlinelibrary.wiley.com/doi/abs/10.1002/er.1397?casa_token=0ClxQHSkd4cAAAAA%3ATgg2BBw7sWLeyuYNJ0iKYYn2dZagGu7KqumU9o4sHIpboZjx7G6EHQW-jChovYatR8utzSTgdnqfva8 PMFC | <ref>[https://onlinelibrary.wiley.com/doi/abs/10.1002/er.1397?casa_token=0ClxQHSkd4cAAAAA%3ATgg2BBw7sWLeyuYNJ0iKYYn2dZagGu7KqumU9o4sHIpboZjx7G6EHQW-jChovYatR8utzSTgdnqfva8 Model of a PMFC Producing Electricity]</ref> | ||
7. Microbial desalination cells (MDCs) | ==== 7. Microbial desalination cells (MDCs) ==== | ||
[[File:MDC Diagram.png|right|MDC Diagram. All Rights Reserved.]] | |||
MDCs use the electric potential difference across the anode and the cathode through MFC technology to operate in situ desalination. They comprise an additional middle compartment for water desalinisation which is partitioned by an anion exchange membrane (AEM) towards the anode and a cation exchange membrane (CEM) towards the cathode. Bacteria on the anode oxidize biodegradable substrates and generate electrons and protons. The electrons are externally transferred to cathode whereas the anions in the desalination compartment migrate to the anode and the cations are transferred to the cathode to maintain charge neutrality and therefore desalination of the middle chamber solution occurs. Also, the migration of ions from the saline water in the middle chamber towards each node increases the conductivity of the anolyte and catholyte and so electrical power production is improved due to higher conductivity and mass transfer<ref name="ref1" />. | MDCs use the electric potential difference across the anode and the cathode through MFC technology to operate in situ desalination. They comprise an additional middle compartment for water desalinisation which is partitioned by an anion exchange membrane (AEM) towards the anode and a cation exchange membrane (CEM) towards the cathode. Bacteria on the anode oxidize biodegradable substrates and generate electrons and protons. The electrons are externally transferred to cathode whereas the anions in the desalination compartment migrate to the anode and the cations are transferred to the cathode to maintain charge neutrality and therefore desalination of the middle chamber solution occurs. Also, the migration of ions from the saline water in the middle chamber towards each node increases the conductivity of the anolyte and catholyte and so electrical power production is improved due to higher conductivity and mass transfer<ref name="ref1" />. | ||
<br clear=all /> | <br clear=all /> | ||
<ref>[https://pubs.acs.org/doi/pdf/10.1021/es901950j MDC Diagram]</ref> | <ref>[https://pubs.acs.org/doi/pdf/10.1021/es901950j MDC Diagram]</ref> | ||
==References== | ==References== | ||
<references /> | <references /> |
Latest revision as of 12:00, 13 January 2021
Bioelectrochemical systems (BESs) are integrated systems combining wastewater treatment with energy production and resource recovery. They utilize microorganisms to biochemically catalyse complex substrates into useful energy products, in which the catalytic reactions take place on electrodes[1].
BESs have boomed over the past decade for their contribution as an emerging sustainable technology for concurrent electricity production and wastewater treatment. In addition, BESs also offer unique possibilities for clean and efficient production of fuels and high-value chemicals using microorganisms. Applications of BESs include: microbial desalination cells (MDCs), sediment/plant microbial fuel cells (MFCs), microbial electrosynthesis (MES) converts CO2 or organic molecules to higher value organic molecules, they are efficient bioreactors for the treatment of pollutants and toxic wastewater (process known as bioelectrochemical treatment (BET)/microbial electroremediation (MER)).
In these electrochemical systems, the redox potentials of an oxidation reaction at the anode and a reduction reaction at the cathode create a potential difference which is the driving force for electrons to flow from a low potential to high potential. This flow of electrons through an external circuit is measured as electric current. Whenever, microbes or enzymes are involved in the oxidation or reduction or both reactions, the system is termed as a bioeclectrocehmical system (BES) or microbial electrochemical system (MXC)[2].
Types of Bioelectrochemical Systems

- Microbial fuel cells (MCFs)
- Microbial electrolysis cells (MECs)
- Microbial electrosynthesis (MES)
- Enzymatic fuel cells (EFCs)
- Microbial solar cells (MSCs)
- Plant microbial fuel cells (PMFCs)
- Microbial desalination cells (MDCs)
1. Microbial fuel cells (MCFs)

MFCs harness electrical current from the microbial oxidation of organic matter using a solid electrode as an electron acceptor. The anode surface facilitates microbial attachment and oxidation of organics, therefore generating electrons which are then simultaneously transferred to the cathode compartment through an external circuit containing an external load. Electroneutrality is warranted by ions transport through an ion-permeable medium or a membrane while electricity is produced in the process.
A number of bacterial species have been identified with the ability to produce electric current. The Shewanella and Geobacter species were earlier found as the electron-transferring microbes and so are well-studied. The presence of Geobacteraceae in the bioanode community often showed high power densities registered in MFCs.
The performance of MCFs relies on electrode material and its structure. Graphite electrodes with a roughened surface have shown to produce higher power densities than flat graphite electrodes. Increasing the surface area of MFC anodes using porous materials such as graphite brush, carbon felt and carbon nanotubes, have shown considerable increase in current density. Higher surface area of electrodes provides a larger surface for bacterial adhesion and electron transfer between the bacteria and electrode. Direct electron transfer phenomenon of microbes with metal electrodes has also been demonstrated including dimensionally stable anodes (DSA, titanium over iridium and tantalum oxide), stainless steel and platinum electrodes. However, current densities obtained with porous carbon anodes are typically higher than those reported with metals except for platinum[2].
[3]
2. Microbial electrolysis cells (MECs)
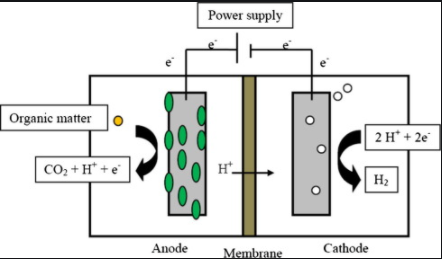
MECs utilize the property of bacteria to convert chemical energy to electrical energy and allow electrolysis of water. External power applied onto the electrical circuit of BES drives electrons from anode to the cathode. This also supports the hydrogen production at the cathode which operates under anaerobic conditions. However the anoxic environment in MECs, along with high concentrations of hydrogen production, can also promote methane production once CO2 and methanogens are available. Methods to mitigate toxic build up includes the aeration of the cathode chamber between batches, lowering of the pH, operation at short retention times, giving a heat shock to the inoculum and adding chemicals that inhibit the growth of methanogens.
In an MEC with bioanode and biocathode, expensive metals such as platinum are not required as catalyst and the enrichment of microbes on the carbon cathode decreases the start-up time and produces comparable current densities to those of bioanode. Also the hydrogen synthesized in MECs can also drive the biochemical production of other chemicals[2].
3. Microbial electrosynthesis (MES)
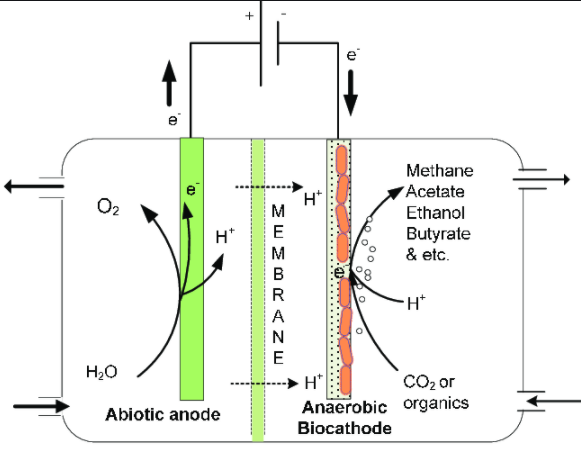
MES (also known as bioelectrosynthesis) uses the reducing power generated from the anodic oxidation to produce value added products at the cathode. Cathodic biocatalysts (with attached cathodic biofilms) reduce the available terminal electron acceptor to produce value added products. Biocathodes are the key components of microbial electrosynthesis, where the electrode oxidizing microorganisms are involved in the formation of reduced value-added product such as acetate, ethanol, butyrate. MES includes the production of chemical compounds in an electrochemical cell by electricity-driven CO2 reduction as well as reduction/oxidation of other organic feedstocks using microbes as biocatalyst[2].
4. Enzymatic fuel cells (EFCs)

EFCs utilize specific enzymes on the electrode surface (or in electrolyte suspension) that facilitate the catalytic oxidation of fuel and drive specific reactions desired for various applications. Different enzymes are used on anode and cathodes, based on their specific redox function. As highly selective enzymes are used at each node, then this eliminates the need of any membrane between the anode and cathode compartments. Enzymatic catalysts usually oxidize the fuel partially and heat generation occurs as a result of side reactions which can impact enzymatic activity. The shelf life of these enzyme-based systems can be extended through encapsulation in micelle polymers that provide a buffering capacity for pH control and a hydrophobic niche to prevent enzymatic degradation[2].
5. Microbial solar cells (MSCs)

MSCs make use of photoautotrophic microbes or higher plants to entrap solar energy which is further utilized by electroactive bacteria to perform electrode-driven reactions. These reactions include generation of electric current or fuels such as hydrogen, methane and ethanol. Primarily photosynthesis leads to the generation of organic compounds, which are subsequently fed into the anode compartment where they are oxidized by electroactive microbes to produce electrons. These electrons are then transferred to the cathodic side where reduction of oxygen leads to the formation of water[2].
6. Plant microbial fuel cells (PMFCs)
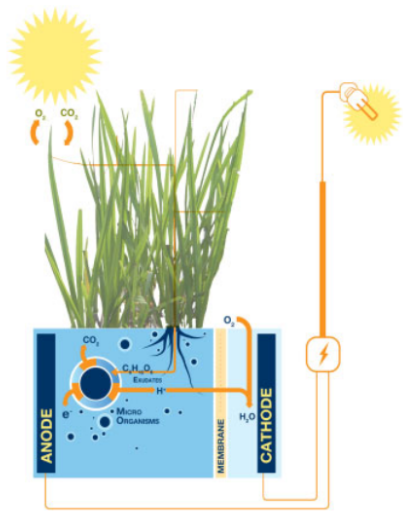
PMFCs incorporate the living plant's root system into the MFC anode. The organic products (rhizodeposits) released to the soil by the roots are used as substrate for electricity production by electroactive microbes. So overall PMFCs harness solar radiation by transforming it into green electricity in a clean and efficient manner. The choice of a plant is important as it directly controls the amount of rhizodeposits for bioelectricity generation. PMFCs from Pennisetum setaceum are the most sustainable in terms of power production and S. anglica PMFCs with integrated oxygen reducing biocathodes reached the highest long-term power output. Reed manna grass, rice plants and Spartina anglica have also been used in separate studies[2].
7. Microbial desalination cells (MDCs)

MDCs use the electric potential difference across the anode and the cathode through MFC technology to operate in situ desalination. They comprise an additional middle compartment for water desalinisation which is partitioned by an anion exchange membrane (AEM) towards the anode and a cation exchange membrane (CEM) towards the cathode. Bacteria on the anode oxidize biodegradable substrates and generate electrons and protons. The electrons are externally transferred to cathode whereas the anions in the desalination compartment migrate to the anode and the cations are transferred to the cathode to maintain charge neutrality and therefore desalination of the middle chamber solution occurs. Also, the migration of ions from the saline water in the middle chamber towards each node increases the conductivity of the anolyte and catholyte and so electrical power production is improved due to higher conductivity and mass transfer[2].
References
- ↑ Sustainable Waste-to-Energy Technologies: Bioelectrochemical Systems
- ↑ 2.0 2.1 2.2 2.3 2.4 2.5 2.6 2.7 2.8 Bioelectrochemical Systems (BESs)
- ↑ MFC Diagram
- ↑ MEC Diagram
- ↑ MES Diagram
- ↑ Schematic of an EFC-based Biosensor and its Detection Mechanism
- ↑ MSC Diagram
- ↑ Model of a PMFC Producing Electricity
- ↑ MDC Diagram